Trigonal pyramidal molecular geometry plays a crucial role in understanding the structure and properties of various molecules in chemistry. This fascinating arrangement occurs when a central atom bonds with three other atoms, leaving one lone pair of electrons. The result? A shape resembling a pyramid with a triangular base, which significantly influences chemical reactions and molecular behavior. Whether you're a student diving into chemistry for the first time or a seasoned professional exploring advanced concepts, this article will demystify the intricacies of trigonal pyramidal geometry and its applications.
What makes trigonal pyramidal geometry particularly interesting is its impact on molecular polarity, bond angles, and reactivity. Unlike tetrahedral or linear geometries, the presence of a lone pair in trigonal pyramidal molecules causes a deviation in bond angles, typically reducing them to around 107 degrees instead of the ideal 109.5 degrees. This unique characteristic is vital for predicting molecular interactions and understanding phenomena like hydrogen bonding and dipole moments. From ammonia (NH₃) to phosphine (PH₃), examples of trigonal pyramidal molecules abound in nature, making this topic both relevant and essential.
In this comprehensive guide, we will delve into the intricacies of trigonal pyramidal geometry, exploring its formation, properties, and real-world applications. We'll also address common questions and misconceptions surrounding this molecular arrangement, ensuring you leave with a thorough understanding of its significance in chemistry. So, whether you're looking to ace your next chemistry exam or deepen your knowledge of molecular geometry, this article has everything you need to know about trigonal pyramidal structures.
Read also:How To Master The Remote Raspberry Pi Iot Tutorial For Beginners
Table of Contents
- 1. What Exactly is Trigonal Pyramidal Geometry?
- 2. How Does Trigonal Pyramidal Geometry Form?
- 3. Why Are Bond Angles Important in Trigonal Pyramidal Structures?
- 4. Can Trigonal Pyramidal Molecules Be Polar?
- 5. Common Examples of Trigonal Pyramidal Molecules
- 6. How Does Trigonal Pyramidal Geometry Affect Chemical Reactivity?
- 7. Applications of Trigonal Pyramidal Molecules in Everyday Life
- 8. Frequently Asked Questions About Trigonal Pyramidal Geometry
- Conclusion
What Exactly is Trigonal Pyramidal Geometry?
Trigonal pyramidal geometry refers to a specific molecular shape where a central atom is bonded to three surrounding atoms, with one lone pair of electrons occupying the fourth position. This arrangement results in a three-dimensional structure resembling a pyramid with a triangular base. Unlike tetrahedral geometry, where all four positions are occupied by bonding pairs, the presence of a lone pair in trigonal pyramidal molecules leads to a deviation in bond angles and overall molecular symmetry.
The concept of trigonal pyramidal geometry is rooted in the VSEPR (Valence Shell Electron Pair Repulsion) theory, which explains how electron pairs around a central atom repel each other to minimize energy and achieve the most stable configuration. In this case, the lone pair exerts a greater repulsive force than bonding pairs, causing the bond angles to contract slightly. This phenomenon is observed in molecules like ammonia (NH₃) and sulfur trioxide (SO₃), where the lone pair influences the molecular shape and properties.
Understanding trigonal pyramidal geometry is essential for predicting molecular behavior, such as polarity, reactivity, and solubility. It also plays a critical role in fields like biochemistry, materials science, and environmental chemistry, where molecular structure directly impacts functionality. By studying this geometry, chemists can design more effective drugs, catalysts, and materials tailored to specific applications.
How Is Trigonal Pyramidal Geometry Different From Tetrahedral Geometry?
While both trigonal pyramidal and tetrahedral geometries involve four electron pairs around a central atom, the key difference lies in the presence of lone pairs. In tetrahedral geometry, all four positions are occupied by bonding pairs, resulting in symmetrical bond angles of approximately 109.5 degrees. In contrast, trigonal pyramidal geometry features three bonding pairs and one lone pair, leading to a reduced bond angle of around 107 degrees due to the stronger repulsion exerted by the lone pair.
This distinction has significant implications for molecular properties. For instance, tetrahedral molecules tend to be nonpolar if all surrounding atoms are identical, whereas trigonal pyramidal molecules often exhibit polarity due to the asymmetrical distribution of charge caused by the lone pair. Additionally, the reduced bond angles in trigonal pyramidal structures can affect molecular interactions, such as hydrogen bonding and van der Waals forces.
How Does Trigonal Pyramidal Geometry Form?
The formation of trigonal pyramidal geometry follows the principles of VSEPR theory, which predicts molecular shapes based on the repulsion between electron pairs around a central atom. When a central atom forms three covalent bonds with surrounding atoms and retains one lone pair, the resulting electron pair geometry is tetrahedral. However, the molecular geometry appears as trigonal pyramidal due to the influence of the lone pair on bond angles and spatial arrangement.
Read also:Is Kat Dennings A Republican Exploring The Actresss Political Views
For example, consider ammonia (NH₃). Nitrogen, the central atom, has five valence electrons. Three of these electrons form covalent bonds with hydrogen atoms, while the remaining two electrons exist as a lone pair. According to VSEPR theory, the electron pairs arrange themselves in a tetrahedral configuration to minimize repulsion. However, the lone pair occupies more space than bonding pairs, causing the hydrogen atoms to move closer together and reducing the bond angle to approximately 107 degrees.
Factors such as electronegativity, atomic size, and the nature of surrounding atoms can also influence the formation of trigonal pyramidal geometry. For instance, larger central atoms or highly electronegative surrounding atoms may result in variations in bond angles and molecular symmetry. Understanding these factors is crucial for predicting and explaining molecular behavior in diverse chemical systems.
What Role Does the Lone Pair Play in Trigonal Pyramidal Geometry?
The lone pair in trigonal pyramidal geometry is a critical determinant of molecular shape and properties. Unlike bonding pairs, which are shared between two atoms, lone pairs are localized on the central atom and occupy more space due to their greater electron density. This increased electron density leads to stronger repulsive forces between the lone pair and bonding pairs, causing the latter to compress and form a trigonal pyramidal shape.
This phenomenon has significant implications for molecular polarity and reactivity. The asymmetrical distribution of charge caused by the lone pair often results in a net dipole moment, making trigonal pyramidal molecules polar. Additionally, the lone pair can participate in hydrogen bonding or other intermolecular interactions, influencing solubility, boiling points, and other physical properties. By understanding the role of lone pairs, chemists can better predict and manipulate molecular behavior in various applications.
Why Are Bond Angles Important in Trigonal Pyramidal Structures?
Bond angles are a critical aspect of trigonal pyramidal geometry, as they directly influence molecular shape, polarity, and reactivity. In an ideal tetrahedral arrangement, the bond angle is approximately 109.5 degrees. However, in trigonal pyramidal structures, the presence of a lone pair reduces this angle to around 107 degrees. This deviation occurs because lone pairs exert greater repulsive forces than bonding pairs, causing the latter to move closer together.
The reduction in bond angles has significant implications for molecular properties. For instance, the asymmetrical arrangement of atoms in trigonal pyramidal molecules often results in a net dipole moment, making them polar. This polarity can affect intermolecular forces, solubility, and chemical reactivity. Additionally, smaller bond angles may lead to increased steric hindrance, influencing reaction rates and pathways. Understanding bond angles is therefore essential for predicting and explaining molecular behavior in various chemical contexts.
Experimental techniques such as X-ray crystallography and spectroscopy are commonly used to measure bond angles in trigonal pyramidal molecules. These methods provide valuable insights into molecular structure and help validate theoretical predictions based on VSEPR theory. By combining experimental data with computational models, chemists can gain a deeper understanding of the factors influencing bond angles and their impact on molecular properties.
How Do Bond Angles Affect Molecular Polarity?
Bond angles play a crucial role in determining the polarity of trigonal pyramidal molecules. Polarity arises from the asymmetrical distribution of charge within a molecule, which is influenced by factors such as electronegativity differences between atoms and molecular geometry. In trigonal pyramidal structures, the presence of a lone pair and the associated reduction in bond angles often result in a net dipole moment, making the molecule polar.
For example, ammonia (NH₃) exhibits polarity due to the difference in electronegativity between nitrogen and hydrogen atoms, combined with the trigonal pyramidal geometry. The lone pair on nitrogen creates an asymmetrical charge distribution, resulting in a net dipole moment. This polarity enables ammonia to participate in hydrogen bonding, a key factor in its high boiling point and solubility in water. By understanding the relationship between bond angles and molecular polarity, chemists can design molecules with specific properties for various applications.
Can Trigonal Pyramidal Molecules Be Polar?
Yes, trigonal pyramidal molecules are often polar due to the asymmetrical distribution of charge caused by the presence of a lone pair. Polarity arises when there is a difference in electronegativity between atoms and an asymmetrical molecular geometry that prevents the cancellation of dipole moments. In trigonal pyramidal structures, the lone pair creates an uneven electron distribution, resulting in a net dipole moment and making the molecule polar.
For instance, ammonia (NH₃) is a classic example of a polar trigonal pyramidal molecule. The nitrogen atom is more electronegative than hydrogen, causing the nitrogen-hydrogen bonds to have partial negative charges. The lone pair on nitrogen further enhances this polarity by creating an asymmetrical charge distribution. This polarity enables ammonia to engage in hydrogen bonding, a key factor in its high boiling point and solubility in water.
However, not all trigonal pyramidal molecules are polar. The polarity depends on factors such as electronegativity differences between atoms, bond angles, and molecular symmetry. For example, boron trifluoride (BF₃) exhibits trigonal planar geometry rather than trigonal pyramidal due to the absence of a lone pair, making it nonpolar. Understanding these factors is essential for predicting molecular behavior and designing molecules with specific properties.
What Factors Influence the Polarity of Trigonal Pyramidal Molecules?
Several factors influence the polarity of trigonal pyramidal molecules, including electronegativity differences, bond angles, and molecular symmetry. Electronegativity differences between the central atom and surrounding atoms determine the distribution of charge within the molecule. Larger differences typically result in greater polarity, as the more electronegative atom pulls electrons closer to itself.
Bond angles also play a critical role in determining molecular polarity. In trigonal pyramidal structures, the presence of a lone pair reduces bond angles, creating an asymmetrical arrangement of atoms that often results in a net dipole moment. Additionally, molecular symmetry can affect polarity, as symmetrical arrangements tend to cancel out dipole moments, while asymmetrical arrangements enhance them.
Common Examples of Trigonal Pyramidal Molecules
Trigonal pyramidal geometry is observed in numerous molecules, many of which play vital roles in chemistry, biology, and industry. Some common examples include ammonia (NH₃), phosphine (PH₃), and sulfur trioxide (SO₃). These molecules exhibit characteristic properties such as polarity, reactivity, and solubility, which are directly influenced by their trigonal pyramidal shape.
Ammonia (NH₃) is perhaps the most well-known trigonal pyramidal molecule, widely used in fertilizers, refrigerants, and industrial chemicals. Its polarity and ability to form hydrogen bonds make it an excellent solvent and a key participant in various chemical reactions. Phosphine (PH₃), another trigonal pyramidal molecule, is used in semiconductor manufacturing and as a fumigant. Sulfur trioxide (SO₃), although technically trigonal planar in its gas phase, adopts a trigonal pyramidal geometry in certain solid forms, influencing its reactivity and applications in sulfuric acid production.
Other examples of trigonal pyramidal molecules include amines, phosphines, and certain oxides. These molecules exhibit diverse properties and applications, underscoring the importance of understanding trigonal pyramidal geometry in chemistry.
What Are Some Industrial Applications of Trigonal Pyramidal Molecules?
Trigonal pyramidal molecules find extensive use in various industrial applications, ranging from agriculture to electronics. Ammonia (NH₃), for instance, is a critical component in fertilizer production, providing essential nitrogen for plant growth. It is also used as a refrigerant in large-scale cooling systems and as a precursor for numerous industrial chemicals.
Phosphine (PH₃) is employed in semiconductor manufacturing to dope silicon wafers, enhancing their electrical conductivity. Additionally, it serves as a fumigant for stored grains and other agricultural products, effectively controlling pests. Sulfur
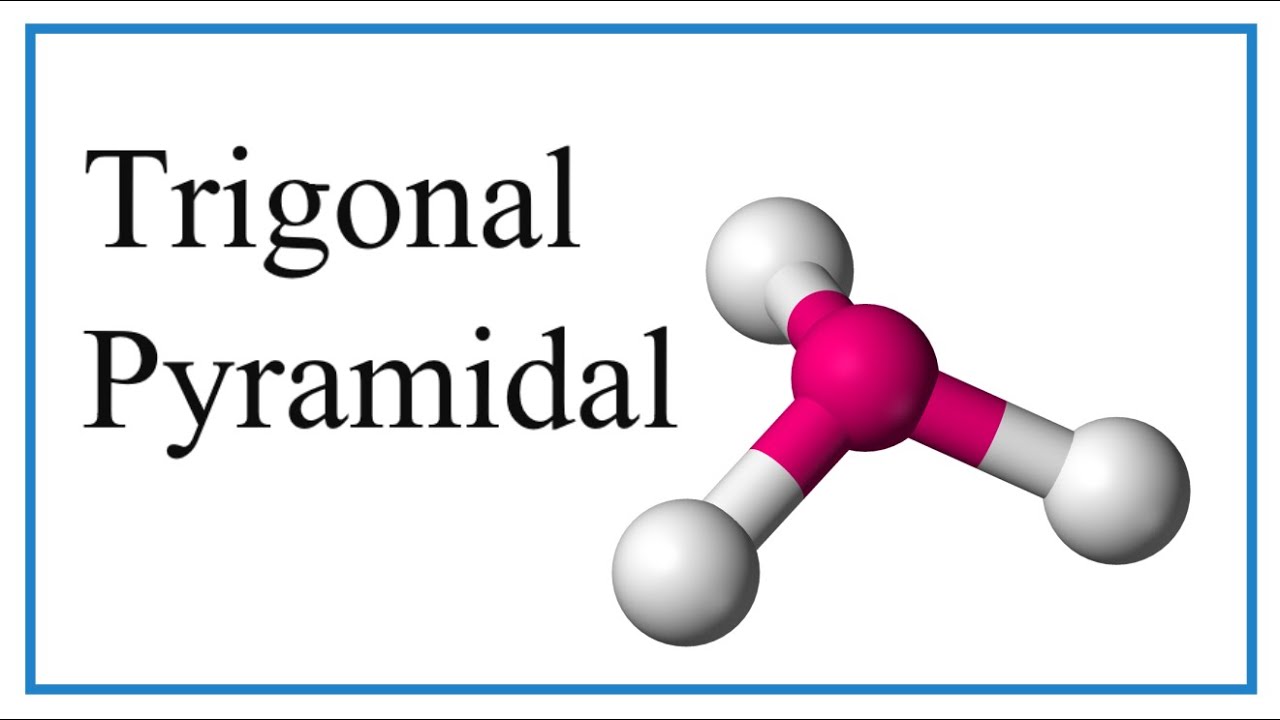

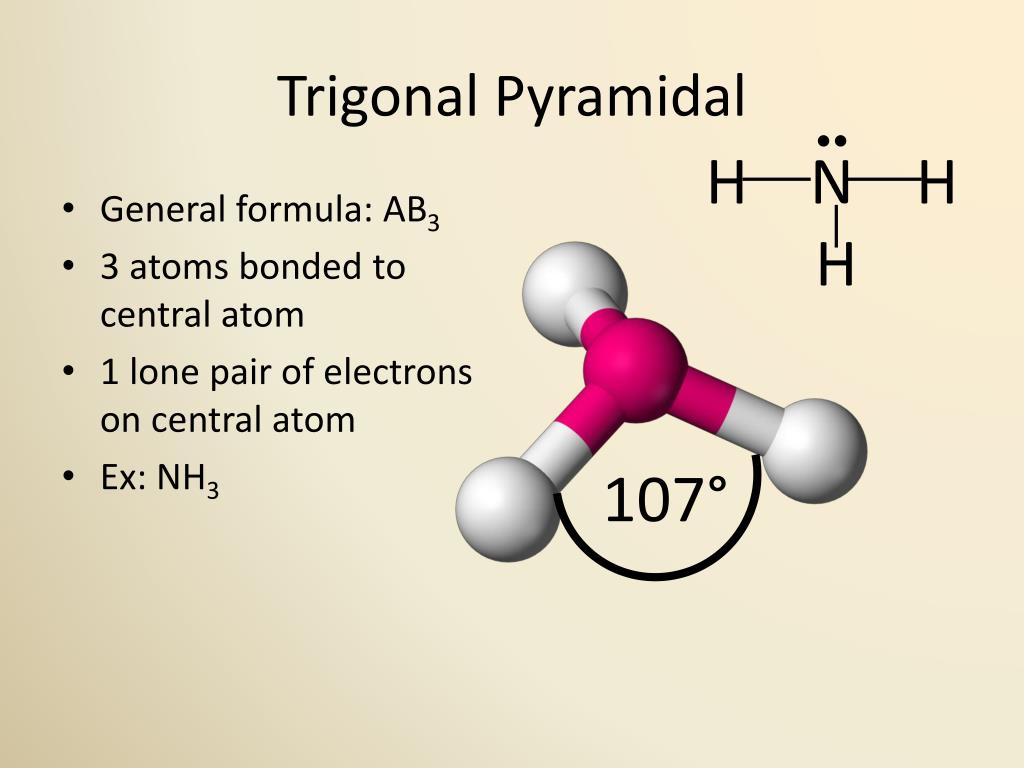